A: The “GNSS Solutions” column in the May/June issue of Inside GNSS [1] described the commitments that have been made by GNSS service providers in terms of limits on Signal-in-Space (SIS) range errors, satellite failure probabilities, and times to alert and recover from failures.
These commitments are important to all GNSS users but especially those whose applications are safety critical. For example, draft standards for the application of Advanced Receiver Autonomous Integrity Monitoring (ARAIM) in civil aviation propose to use these commitments to establish the Integrity Support Message (ISM) parameters needed as inputs to the ARAIM algorithm implemented on board each aircraft.
The values expressed in the service provider commitments tend to be conservative because they cannot easily or rapidly be updated to reflect changes in constellation behavior [1]. Thus, the promised values need to exceed (with margin) both typical error variations and potential future deterioration in satellite and/or ground segment performance over time, even if this is thought to be unlikely. While GPS is a mature constellation with well-understood performance, this is not necessarily the case for other GNSS constellations that are either relatively new (Galileo) or in the process of modernization (Beidou, GLONASS).
The projected availability of ARAIM for civil aviation suffers from this conservatism, but it is acceptable given civil ARAIM will incorporate multiple GNSS constellations in user position solutions and thus obtain sufficient satellite and constellation redundancy. Civil ARAIM will be implemented first for flight phases requiring only 2D horizontal positioning assurance (“horizontal ARAIM” or “H-ARAIM”) [1]. Examples of the protection levels and availability achievable by combining GPS and Galileo are shown in Figure 2 of [2]. Once certified and demonstrated in flight operations, ARAIM for the more-demanding flight phases requiring vertical position assurance (“V-ARAIM”) is expected to follow.
Research on ARAIM’s benefits for other classes of users is also progressing but must consider different mission constraints. One example is U.S. military users who will use GPS M-code signals on L1 and L2 as the primary (and perhaps) only sources of satellite positioning. Furthermore, the missions of greatest interest to the military require at least V-ARAIM levels of accuracy and integrity assurance. To meet these objectives, this article shows how additional ground monitoring for GPS and other GNSS constellations can improve upon the commitments shown in [1] and provide useful performance for military users of GPS and other multi-constellation users of V-ARAIM.
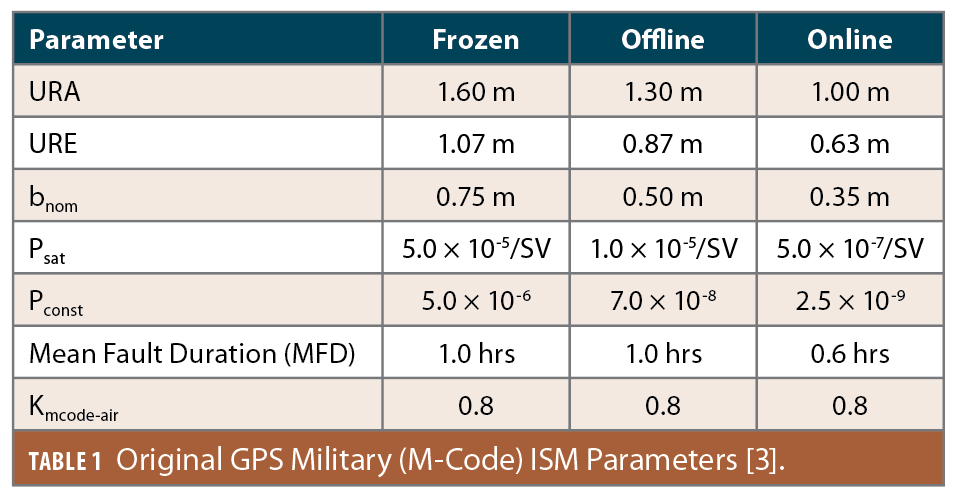
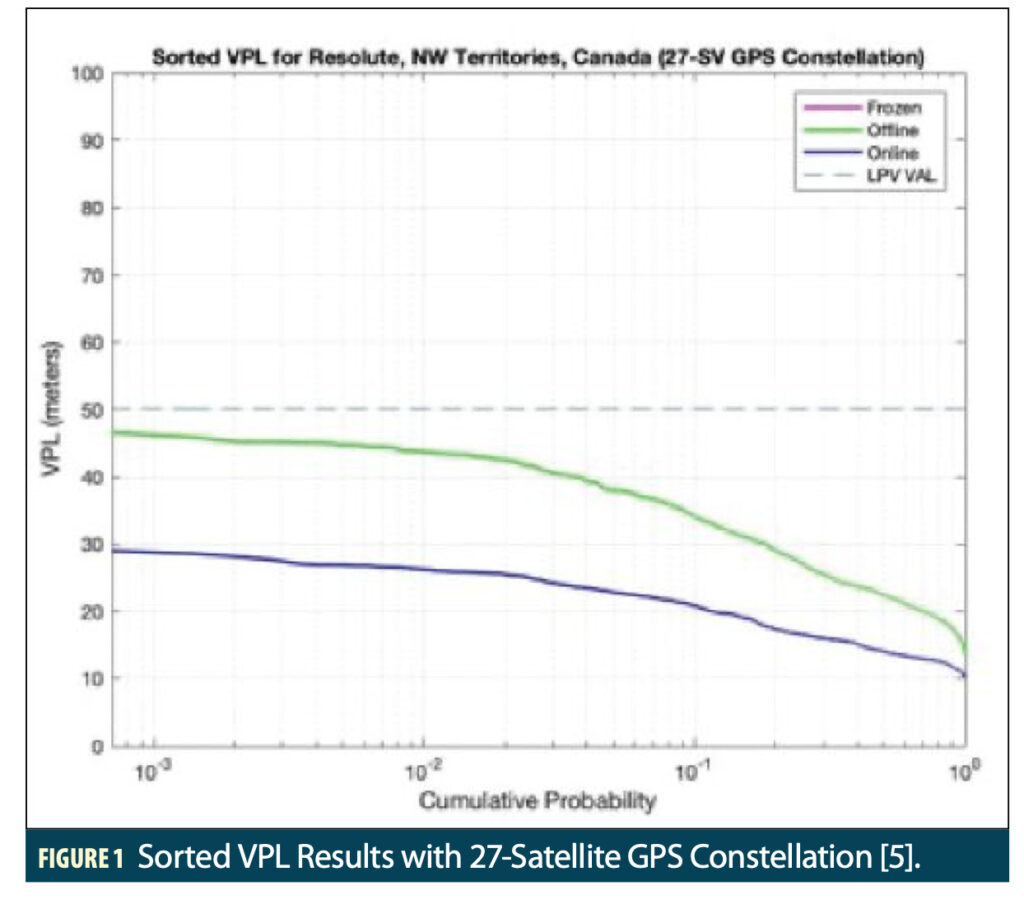
ISM Parameter Estimates for Military ARAIM and MAAST Results
Prior to implementing specific ground monitoring techniques, the authors evaluated the effectiveness of V-ARAIM for military users of GPS using estimated ISM parameters that reflected the use of GPS M-code on L1 and L2 and ISM parameter updates made more frequently than now foreseen for civil ARAIM. These values are shown in Table 1 (from [3]) using the ISM parameter definitions in [1, 3] and an earlier “GNSS Solutions” column in the January/February 2021 issue of Inside GNSS [4]). The three columns of Table 1 correspond to three different protocols for updating ISM parameters as follows:
Frozen: This represents, at most, very infrequent ISM parameter updates. The civil ARAIM approach of relying on constellation commitments is an example of this, as its ISM parameters would (most likely) only change if or when the commitments themselves are updated in constellation standards and reports to the International Civil Aviation Organization (ICAO) [1]. Planned updates on a roughly yearly basis also fall in this category.
Offline: This represents ISM parameter updates (or at least the capability to update when needed) on a regular basis from quarterly to monthly to weekly. Offline ground monitoring would be the basis for generating any updates or confirming the current ISM parameters are appropriate.
Online: This represents frequent ISM parameter updates every hour or every few hours. The ground monitoring responsible for these updates would need to provide updated estimates of satellite and constellation error states on a minute-by-minute basis.
There are several differences between this table and the GPS constellation commitments in Table 1 of [1]. First, GPS commits to the URA included in the broadcast navigation data message at any given time, whereas a fixed number (meant to include almost all scenarios and times) is shown here. User Range Error (URE) is also shown here and represents a typical (not necessarily bounding) one-sigma error value, which is why it is significantly lower than URA.
A maximum bias term (bnom) is also included here and represents a not-to-exceed mean error in the (assumed Gaussian) SIS range error distribution. Finally, Kmcode–air is a multiplier that is applied to the RTCA standard model for airborne user range error (σair) to represent the lower noise and multipath error obtained from using M-code in place of C/A code [4]:

More rapid ground monitoring and ISM parameter updates are the key to providing less-conservative numbers that result in better user performance and availability. That is why the parameters in Table 1 improve when moving rightward from Frozen to Offline to Online ISM alternatives.
The impact of these improvements on V-ARAIM availability of the civilian “Localizer Performance with Vertical Guidance” (LPV) low-visibility approach operation is shown in Figures 1, 2 and 3 for a single location near Resolute in the Northwest Territories of Canada at 74.7o North, 94.9o West longitude[5].
LPV integrity requires that Vertical and Horizontal Protection Levels (VPLs and HPLs) be computed in real time that bound actual errors in these position axes to a probability of 1−10-7 per approach (i.e., exceeding either bound is allowed only once in 10 million approaches). For an LPV approach to be available, VPL must be below a 50-meter Vertical Alert Limit (VAL), while HPL must be no greater than a Horizontal Alert Limit (HAL) of 40 meters.
Figure 1 shows results when using only the 27-satellite “expandable” GPS constellation given in Section 3.2 of the most recent GPS SPS Performance Standard (GPS SPS PS) [6]. Each line in the plot is based on Vertical Protection Level values as generated by the Stanford MAAST software tool.
Each 1-minute time step in a 24-hour day of repeatable GPS satellite geometries has a particular VPL derived from the “snapshot” V-ARAIM algorithm [2, 5]. Once all epochs have been analyzed, the vector of VPL versus time is sorted from smallest (on the right) to largest (on the left) and plotted as shown. The x-axis represents the cumulative distribution of VPL and makes it easy to identify the probability (or percentage of simulated time epochs) in which VPL exceeds a particular y-axis value. The horizontal line at y=50 meters represents the VAL for LPV, which is the most constraining requirement on LPV availability.
Figure 1 shows that use of the Online ISM parameters in V-ARAIM (the dark blue line) results in VPLs that do not exceed 30 meters and thus stay well below the 50-meter VAL. VPLs for the Offline ISM parameters (the green line) also stay below VAL but come close to it (note about 1% of epochs have VPLs above 45 meters), meaning that relatively little margin exists for slightly worse ISM parameters or satellite outages. A pink line showing VPLs for Frozen ISM parameters is missing from Figure 1 because the ISM value for Pconst for the Frozen case in Table 1 is 5 × 10-6, which makes it impossible to generate error bounds valid to a probability of 1−10-7 per approach, as required for LPV. Thus, the Frozen ISM parameters in Table 1 do not support V-ARAIM for LPV unless they are improved upon.
Figure 2 uses the same approach as Figure 1 but adds the baseline 24-satellite Galileo constellation described in Annex C of the Galileo Open Service: Service Definition Document (Galileo OS SDD) [7]. In this analysis, Galileo satellites use the same ISM parameter values as shown in Table 1 except for the different Psat and Pconst values shown in Table 2.
In the use case of Figure 2, the added Galileo satellites are not used as individual ranging sources within ARAIM as GPS satellites are. They are instead used only as a “constellation check,” meaning an independent position solution is derived using Galileo satellites and Open-Service signals (E1 and E5a) only for comparison to the solution derived using GPS L1 and L2 M-code [5]. This strategy provides a means to detect GPS constellation failures and thus allows use of the Frozen ISM parameters in Table 1.
The results for the Frozen ISM parameters in Figure 2 (the pink line) are not nearly as good as those for the Offline and Online parameters, but the probability of VPL being below VAL is about 95%. The Offline result in Figure 2 is only marginally improved compared to Figure 1, while the Online result is not improved.
Figure 3 uses the same GPS and Galileo satellites as Figure 2, but in this scenario, the Galileo satellites are used as individual ranging sources in the same manner as GPS satellites. In other words, full use is made of the measurement redundancy provided by the Galileo satellites within ARAIM. As a result, the performance of all three ISM parameter alternatives is much better than in Figure 2, as VPL is well below the LPV VAL in all cases. For the Online ISM parameters, VPLs on the order of 10 meters are achieved. This is an idealized use case for U.S. military users because it treats Galileo Open Service (public) signals as equivalent to and interchangeable with GPS M-code signals, but it represents an upper bound on what is possible from use of the Galileo OS.

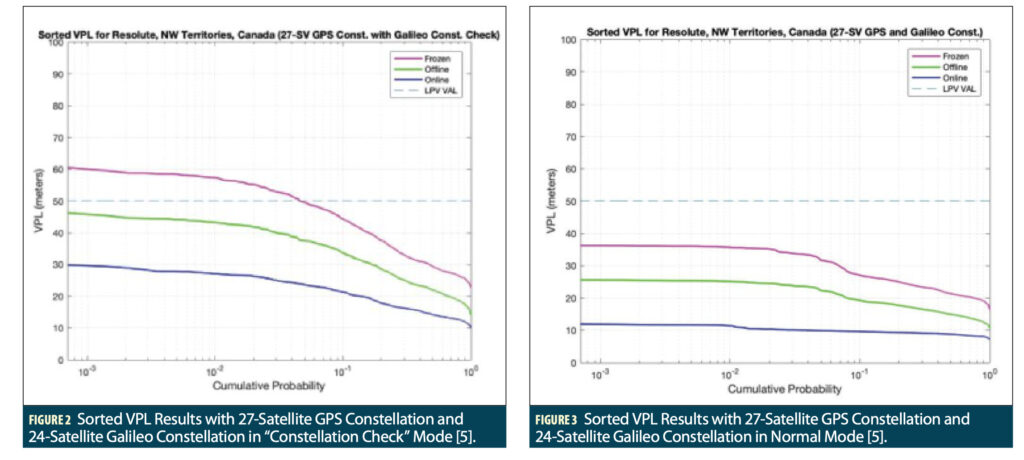
Ground Monitoring Approaches
The ISM parameter values in Tables 1 and 2 represent initial estimates of what could be achieved by additional ground monitoring of GPS and Galileo with varying response times. More recent work [5] has examined the capability of “SBAS-like” online ground monitoring to achieve the 1-meter Online URA value shown in Table 1. “SBAS-like” monitoring refers to the use of monitoring techniques similar to those used in SBAS that generate independent (snapshot) results every epoch. These algorithms can be simulated using the WAAS version of MAAST that is also maintained by Stanford.
The key difference between SBAS-like monitoring in support of ARAIM and SBAS itself is SBAS must generate new estimates of satellite health and be able to provide them to users within the 6-second Time to Alert (TTA) that applies to LPV approaches. Ground monitoring in support of ARAIM does not need to update the ISM this quickly, as the user’s ARAIM algorithm is relied upon to detect new failures within the TTA. The “snapshot” feature of SBAS-like monitoring makes it very suitable for use with Online ISM updates, but other approaches using batch fits or sequential filtering of measurements over time have been proposed and are also being considered [8, 9, 10].
To provide continuous worldwide GNSS constellation monitoring, a worldwide network of ground monitor stations is needed. Figure 4 shows the network of 33 stations used to evaluate SBAS-like monitoring in this work ([5], using original graphic from [11]). It combines the 16 stations used for GPS satellite monitoring by the GPS Control Segment (dark blue and purple circles) with an additional 17 locations (light blue circles) chosen by the authors to minimize gaps in worldwide coverage.
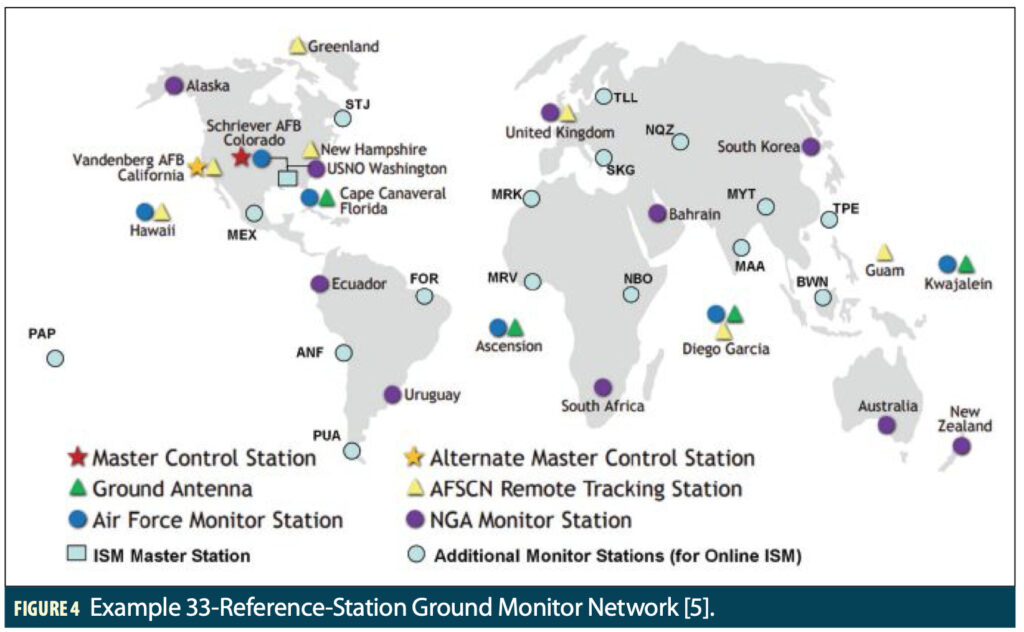
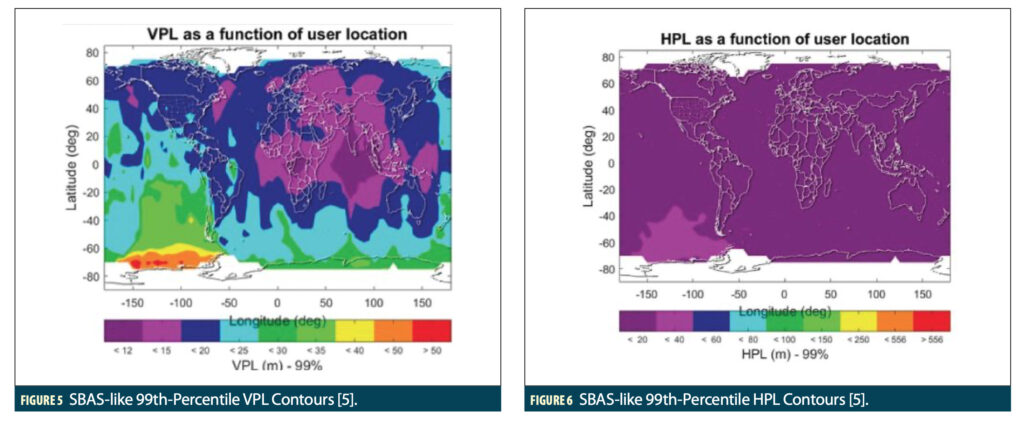
SBAS-like Monitoring: Performance Results
Figures 5 and 6 show contours of VPL and HPL, respectively, based on dual-frequency (L1 and L2) SBAS-like worldwide ground monitoring for the baseline 24-satellite GPS constellation from [6] and the ground station locations in Figure 4 [5]. The WAAS version of MAAST generates SBAS-like VPL and HPL results for each 5-minute epoch at user locations separated by 5 degrees in latitude and longitude spread over the Earth. The contours are drawn by Matlab around the 99th-percentile VPLs and HPLs at each user location (i.e., the PLs in the contours are exceeded on only 1% of epochs for each location).
The PLs in Figures 5 and 6 are generated by the SBAS MOPS technique instead of by ARAIM and thus are not representative of ARAIM user performance. They are included to show the geographic distribution of error bounds as a function of location on Earth, which is driven by satellite geometry and the locations of the 33 ground stations in Figure 4. Regions where ground station coverage is limited, such as the southeastern Pacific Ocean, have higher 99th-percentile VPLs. 99th-percentile HPLs are below 20 meters almost everywhere except in this region of limited coverage over the southern Pacific.
The most important result of SBAS-like simulations for the ARAIM ISM is the one-sigma bound on satellite clock and ephemeris error in the range domain, which is denoted as σUDRE (“sigma User Differential Range Error”) and is equivalent to σDFRE (“sigma Dual Frequency Range Error”) when using dual-frequency ranging to remove ionospheric delay as done here. Figure 7 shows contours of 99th-percentile σUDRE over the world (at locations corresponding to points directly below satellite positions) along with white stars indicating the ground station locations.
The presence of these stars shows where ground monitor coverage is relatively good or poor, and it is not surprising that lower σUDRE values correlate closely with ground station density. In particular, the well-covered Northern Hemisphere (except for the northern Pacific Ocean) has 99th-percentile σUDRE values below 0.9 meters, whereas the least-well-covered locations south of Australia in the Southern Ocean have values approaching 1.4 meters.
Figure 8 shows a histogram of σUDRE values over all satellite locations. The global mean value of σUDRE is about 0.93 meters, while the 90th and 99th percentiles of σUDRE are about 1.1 and 1.26 meters, respectively. These results from SBAS-like monitoring are comparable to what has been assumed for the Online ISM protocol. In particular, a 1-meter URA (along with a maximum bias of ± 0.35 meters) can be supported with confidence in the regions of Figure 7 that are colored dark green and lower in the spectrum diagram on the right-hand side of Figure 7.
Summary and Ongoing Work
This article examines the use of ARAIM for military users with ISMs that are updated more frequently than the commitments offered by GNSS constellation service providers. Frequent ISM parameter updates would be based on continuous ground monitoring that determines error bounds for each satellite on an hourly or daily basis rather than relying on conservative estimates. An evaluation of a global network of ground monitor stations using SBAS-like techniques shows that, with rapid (Online) ISM updates, URA values of 1 meter or less can be supported over most of the northern hemisphere of the globe almost 100% of the time, providing ARAIM performance for military and other users that significantly exceeds the requirements of the LPV approach operation for civil aviation.
Several extensions of this work are being pursued. One is to investigate the usefulness of ground monitoring algorithms not based on SBAS that filter satellite error state estimates over time rather than solving for them independently at each epoch [8, 9, 10]. These are expected to be less burdensome than an SBAS-like approach because they could update and share measurements over minutes instead of seconds. Also, while military use of GPS M-code is one specific example, many other civil users may benefit from more rapid ISM parameter updates and the lower error bounds they make possible.
Acknowledgments
The authors would like to thank Todd Walter, Juan Blanch and many others in the Stanford University GNSS laboratory for their support of this work and their development of the fundamentals of ARAIM for civil aviation on which it rests. They would also like to thank the technical and financial support provided by Mark Crews, Bob Jackson and others at Lockheed Martin.
References
(1) “GPS Solutions” column, Inside GNSS, Vol. 17, No. 3, May/June 2022, pp. 26-33. https://lsc-pagepro.mydigitalpublication.com/publication/?m=61061&i=747851&p=26&ver=html5
(2) J. Blanch, T. Walter, et al., “Baseline Advanced RAIM User Algorithm: Proposed Updates,” Proc. ION ITM 2022, Long Beach, CA, January 2022. http://web.stanford.edu/group/scpnt/gpslab/pubs/papers/blanch_ION_ITM_2022_ARAIM.pdf
(3) A. Katz, S. Pullen, et al., “ARAIM for Military Users: ISM Parameters, Constellation-Check Procedure and Performance Estimates,” Proc. ION ITM 2021, January 2021. http://web.stanford.edu/group/scpnt/gpslab/pubs/papers/Katz_ION_ITM2021_Military_ARAIM.pdf
(4) “GPS Solutions” column, Inside GNSS, Vol. 16, No. 1, Jan./Feb. 2021, pp. 18-23. https://lsc-pagepro.mydigitalpublication.com/publication/?m=61061&i=690698&p=18&ver=html5
(5) S. Pullen, S. Lo, et al, “Ground Monitoring to Support ARAIM for Military Users: Alternatives for Rapid and Rare Update Rates,” Proc. ION GNSS+ 2021, St. Louis, MO, Sept. 2021. http://web.stanford.edu/group/scpnt/gpslab/pubs/papers/Pullen_IONGNSS_2021_Mil_ARAIM.pdf
(6) GPS Standard Positioning Service (SPS) Performance Standard (GPS SPS PS), Washington DC, U.S. Dept. of Defense, 5th Edition, April 2020. https://www.gps.gov/technical/ps/2020-SPS-performance-standard.pdf
(7) Galileo – Open Service—Service Definition Document (Galileo OS SDD), European Union Agency for the Space Programme (EUSPA), Issue 1.2, Nov. 2021. https://www.gsc-europa.eu/sites/default/files/sites/all/files/Galileo-OS-SDD_v1.2.pdf.
(8) Y. Zhai, S. Kiarash, et al., “A Dedicated ARAIM Ground Monitor to Validate the Integrity Support Message,” Proc. ION GNSS+ 2017, Portland, OR, Sept. 2017. https://doi.org/10.33012/2017.15175.
(9) J. Patel, Y. Zhai, et al., “Prototyping an ARAIM Offline Ground Monitor Using Experimental Data,” Proc. ION GNSS+ 2018, Miami, FL, Sept. 2018. https://doi.org/10.33012/2018.16023.
(10) K. Gunning, T. Walter, D. Powell, “SIS Monitoring for ARAIM in the Absence of Precise Clock Estimates,” Proc. ION Pacific PNT 2019, Honolulu, HI, April 2019. http://web.stanford.edu/group/scpnt/gpslab/pubs/papers/Gunning_SIS_Monitoring_2019PacificPNT.pdf.
(11) GPS: The Global Positioning System (U.S. Government website). https://www.gps.gov/multimedia/images/GPS-control-segment-map.pdf.
Authors
Sherman Lo is a senior research engineer at the GPS laboratory at Stanford University. He also is executive director of the Stanford Center for Position Navigation and Time (SCPNT) and a Stanford instructor. His research work focuses on navigation safety, security and robustness. At Stanford, he was Associate Investigator for the FAA evaluation of enhanced Loran and alternative position navigation and timing (APNT) systems for aviation. He currently leads work examining GNSS interference and spoofing detection and mitigation. He has over 130 conference, 28 journal, 14 magazine publications and 8 issued U.S. patents.
Alec Katz is an undergraduate student at Stanford University studying aero/astro engineering. He is currently taking time off from his studies at Stanford to pursue Curious Cardinals, an EdTech startup that helps middle and high school students discover and pursue their passions with the guidance of college mentors. After teaching his first workshop on the future of aviation to high school students at the start of the pandemic, Alec mobilized his most ambitious peers at Stanford and beyond to share topics they were passionate about to K-12 students. Since then, Curious Cardinals has been featured on CNN and in Forbes. Curious Cardinals has now raised $6.8 million in seed funding and Alec was recognized in the 2022 Forbes 30 Under 30 Education and Youngest category. As an aerospace engineer at Stanford, Alec conducted research at the GPS lab and was involved in the solar car team and student space initiative.