SAM PULLEN STANFORD UNIVERSITY, FRANCESCO RISPOLI,
ALESSIA VENNARINI, ARIANNA PERSIA, RADIOLABS , ALESSANDRO NERI, ROMA TRE UNIVERSITY AND RADIOLABS, ROBERTO CAPUA SOGEI
A: Many augmentations of GNSS satellite navigation have been developed and fielded since the GPS constellation was completed and declared fully operational in 1995. The best known of these are satellite-based and ground-based augmentation systems (SBAS and GBAS), which are public systems designed to support civil aviation.
The corrections and integrity information broadcast by SBAS and GBAS are available to all GNSS users with the necessary equipment, but relatively few users beyond aviation have incorporated them into their applications (although this is slowly changing). In addition, many private augmentation systems now provide precise point positioning (PPP) and/or real-time kinematic (RTK) corrections to support other applications requiring sub-meter accuracy. Examples of these applications include surveying, mining and intelligent agriculture.
A growing subset of GNSS applications have demanding safety requirements in addition to needing sub-meter accuracy. These include railway, maritime, automobile and unmanned aerial vehicle (UAV) applications in which passengers and other people are potentially at risk. Specialized GNSS augmentation systems are needed to meet these requirements, and in many cases, multiple different types and sources of augmentation must be combined.
This article describes one example of a “multi-tier” GNSS augmentation system known as the High Integrity EGNSS Layer for Multimodal Eco-friendly Transportation, or HELMET [1] (EGNSS stands for “European Global Navigation Satellites System, although HELMET is not limited to European applications). HELMET is designed to support multiple Service Levels (SLs), where each SL satisfies a set of requirements linked to specific rail and automotive applications. Depending on the SL for a particular user class, HELMET applies different combinations of corrections and user algorithms to maximize performance and availability.
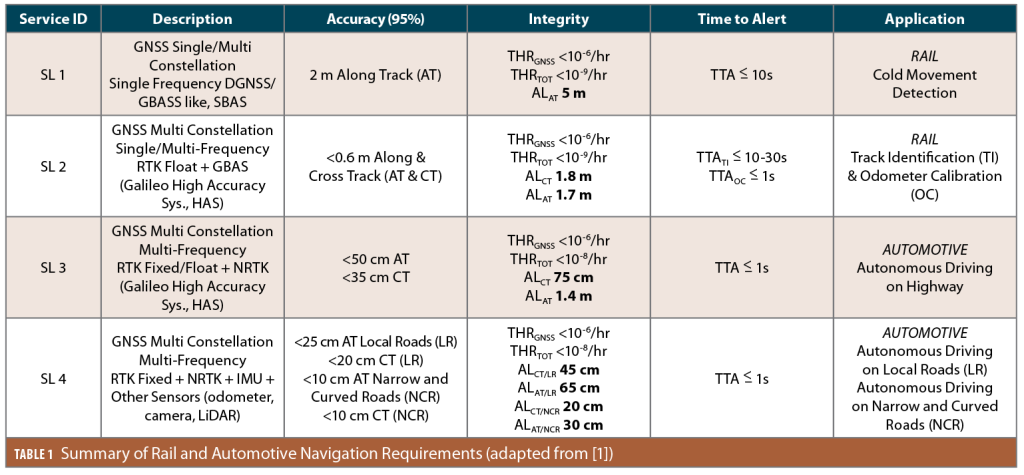
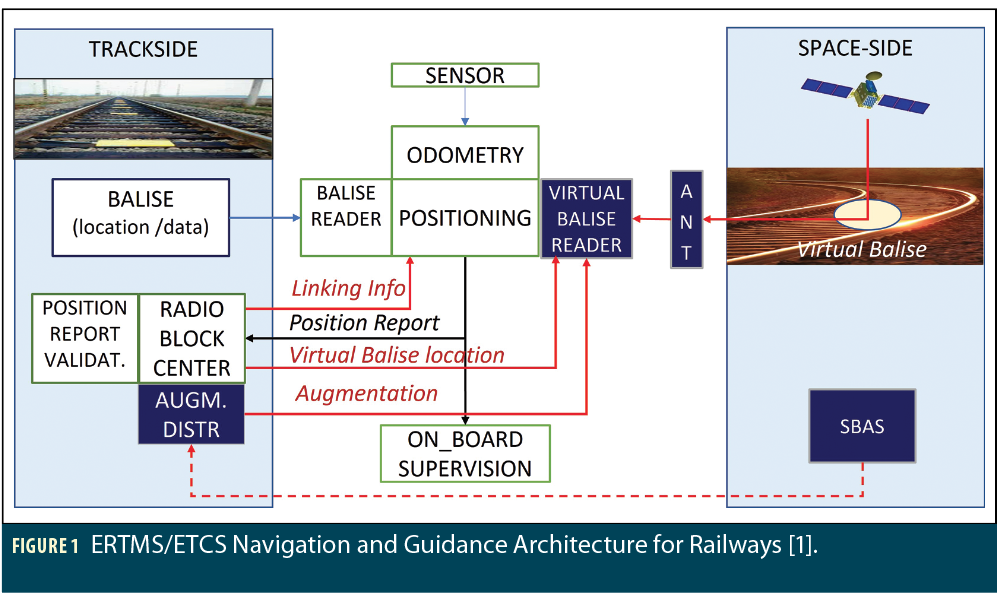
Requirements for Rail and Automotive Navigation
Table 1 summarizes the requirements and corresponding rail, automotive and UAV applications for four different SLs defined for HELMET. These requirements are specified in terms of 95% accuracy in along-track (AT) and cross-track (CT) position axes, integrity alert limits (ALs), tolerable hazard rates (THRs) of loss of integrity (LOI), and times to alert (TTAs) within which anomalies potentially leading to LOI are alerted or (preferably) detected and excluded. LOI occurs when the specified ALs are exceeded in one or more position axes without fault exclusion or annunciation within the TTA. THRs represent the maximum tolerable probability of LOI per exposure interval.
Figure 1 illustrates one of the key railway applications supported by HELMET SLs 1 and 2, which is known as the European Rail Traffic Management System (ERTMS), within which lies the European Train Control System (ETCS) [1,2,3]. ERTMS uses balises placed at known locations along a pre-surveyed track. When a train passes over a balise or a group of closely-spaced balises (provided for redundancy), it receives the balise transmission and obtains its precise location along the track from a database that includes the identifiers and locations of all balises. In between balise groups, the train uses odometry to estimate how far it has progressed along the track. The confidence interval on 1-D train position used to ensure safe separation of trains along the track grows predictably between balise groups and resets itself to a small value when the next balise group is encountered.
While ERTMS is certified and is in operation today, the expense of fielding and maintaining balise groups every few hundred meters along ERTMS-equipped tracks motivated the search for a less expensive variation that uses augmented GNSS to replace physical balises with virtual ones. A GNSS-equipped train knows when it has reached a virtual balise when its GNSS-based location matches its existing database of balise locations. In this way, GNSS can be introduced into ERTMS without otherwise changing the way ERTMS works today or the communication protocols between trackside controllers and individual trains. The Railway High Integrity Navigation Overlay System (RHINOS) project demonstrated the practicality of this approach to GNSS-enabled ERTMS in 2017 [4], and HELMET extends it to additional rail applications (such as track identification) along with automotive and UAV applications.
The existence of high-integrity systems like ERTMS in the rail domain means requirements for GNSS-based rail navigation can be derived from existing standards. The same is not yet true for automotive applications such as driver assistance or autonomous driving. The automotive requirements shown for SLs 3 and 4 in Table 1 are not yet standardized but serve as a guide to the eventual requirements on GNSS augmentation in support of vehicle navigation [5].
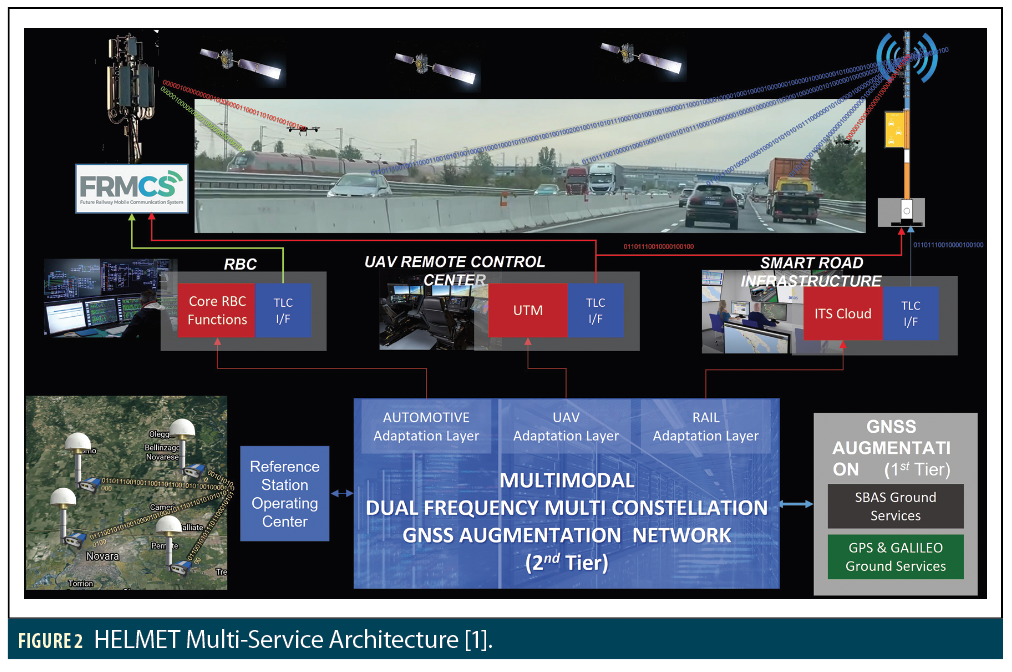
HELMET Multi-Service Architecture
Figure 2 shows a system-level depiction of HELMET that simultaneously supports rail, automotive and UAV applications. A key architectural feature is that GNSS augmentation used within HELMET is broken down into two tiers. The first tier represents SBAS, which is both provided to users via the datalinks built into HELMET (so users are no longer dependent on SBAS corrections transmitted from Geosynchronous satellites) and used as inputs to HELMET’s own integrity monitoring in its Augmentation and Integrity Monitoring Network (AIMN), which is part of the second tier. AIMN consists of a network of reference receivers at fixed sites in the manner of SBAS but with typical separations between receivers of several tens of kilometers instead of hundreds of kilometers.
The second tier of augmentation in Figure 2 also includes separate “adaptation layers” for rail, automotive and UAVs. These layers convert the GNSS corrections and integrity information provided by AIMN into messages specifically designed for different types of users. For example, ERTMS supporting rail applications has existing subsystems such as Radio Block Centers (RBCs) and communication protocols that will be retained. UAV and automobile users are expected to need adaptation to support future messaging and control protocols created for these domains.
Using GNSS augmentation to support multiple user classes is a key HELMET feature and is motivated by economics and efficiency. To meet the requirements of HELMET SLs beyond SL 1, local or regional differential corrections and integrity monitoring provided by the second tier are required. This requires a network of reference receiver sites designed for very high integrity, continuity and availability. Deploying and maintaining these sites and the communications from them to the Reference Station Operating Center (RSOC) shown in Figure 2 represents a significant expense that can and should be shared with multiple user classes.
The functions performed by AIMN within HELMET’s second tier are shown in Figure 3. AIMN applies the reference station measurements collected by the RSOC to calculate code-phase (DGNSS) and carrier-phase corrections (RTK/NRTK/PPP) to support multiple service levels. In parallel, the reference station measurements and SBAS information from the first tier are combined to detect and exclude reference station measurement faults and any remaining Signal-in-Space (satellite and atmospheric) faults not already detected by SBAS. In particular, the smaller separations between HELMET reference stations aids detection of anomalous gradients in ionospheric and tropospheric delays that can create significant errors in DGNSS, PPP and RTK corrections. The resulting corrections are broadcast using RTCM SC-104 and (pending) SC-134 message formats to users and to the adaptation layers that format this information for different user classes.
The subsystems and functions of the train’s on-board units (OBUs) are shown in Figure 4. Sensors include augmented GNSS as well as inertial measurement units (IMUs) and cameras and/or LiDAR to provide an independent representation of the track ahead and the terrain to the sides. GNSS fault detection and exclusion (FDE), including advanced (solution-separation-based) RAIM (ARAIM), occurs before the surviving measurements are combined (“fused”) with those from the other sensors, followed by a second FDE stage in which the redundancy created by multiple sensors is exploited to check for unusual errors in any individual sensor.
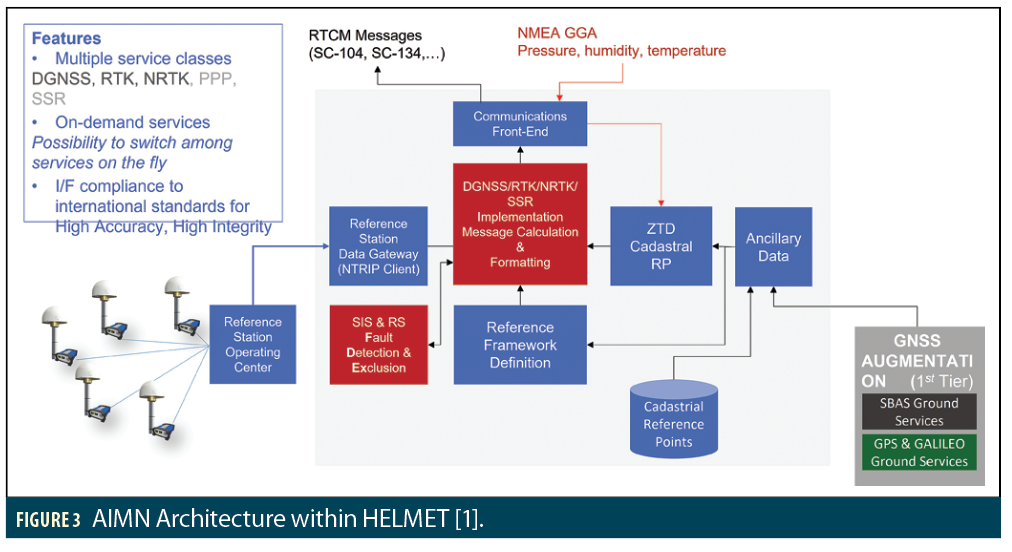
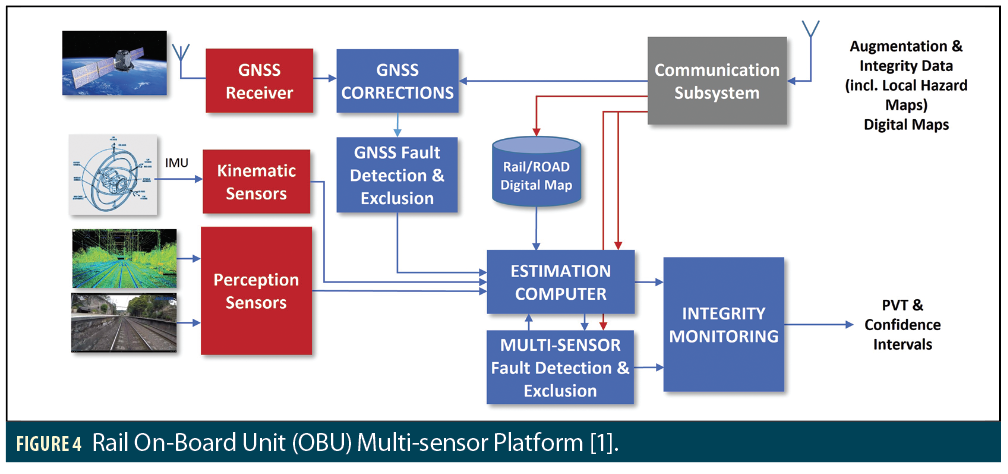
Proof-of-Concept Test Results
Several trials of a proof-of-concept (PoC) HELMET architecture have been conducted to evaluate performance and demonstrate the feasibility of HELMET supporting rail and automotive applications. The first one described in this article was conducted in April 2022 along the track and nearby road between central Rome and Leonardo da Vinci-Fiumicino International Airport (FCO) [1]. As shown in Figure 6, a highway (A51) runs along the same route roughly parallel to the track. This allows for testing the user algorithms in the automobile shown in Figure 7 rather than on an active train, which is harder to equip and collect data from. In this PoC architecture, the first tier of augmentation is provided by EGNOS (the European SBAS), and the second tier is made up of eight reference stations in central Italy that are part of the GNSS R&D Network (GRDNet) deployed and operated by SOGEI, the technology partner of the Italian Ministry of Economy and Finance.
While, in general, automotive and rail OBUs are likely to use different elements of the GNSS corrections provided by AIMN, the automotive OBU for this test was designed to emulate along-track rail navigation as much as possible. For example, automobile OBUs cannot make use of the knowledge of the surveyed track location exploited by train OBUs. To remove this limitation, the automobile OBU was provided with a digital map of the road lane centerlines constructed separately using post-processed RTK measurements. The automobile was kept as close as possible to the center of the lane by careful driving and lane-keeping assistance. Navigation errors were estimated by differencing a RTK-based truth solution from a separate source (not the same as the source of lane centerlines) from the real-time automotive OBU position estimate.
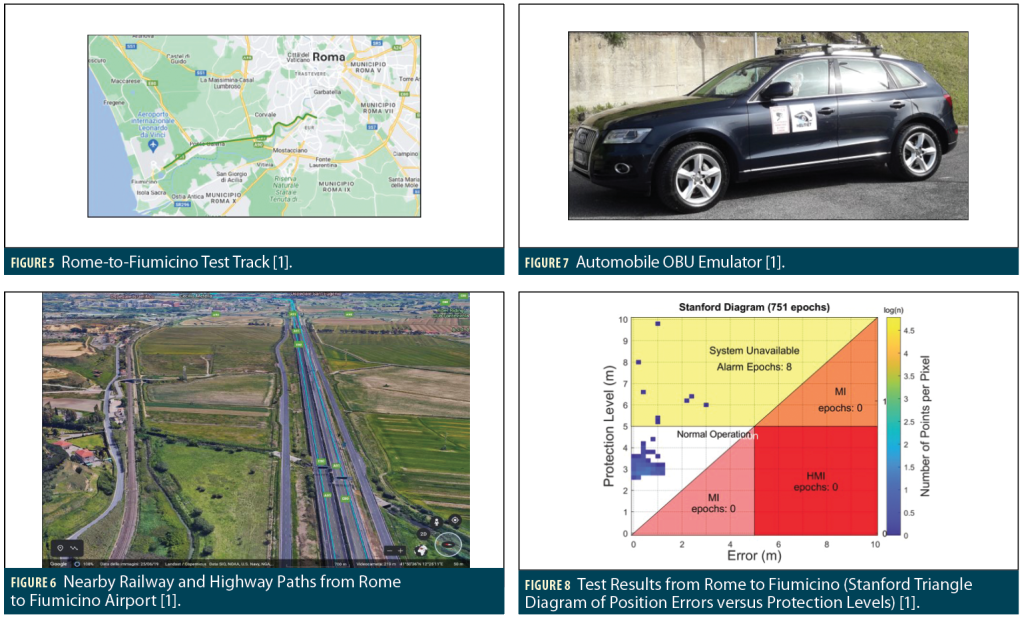
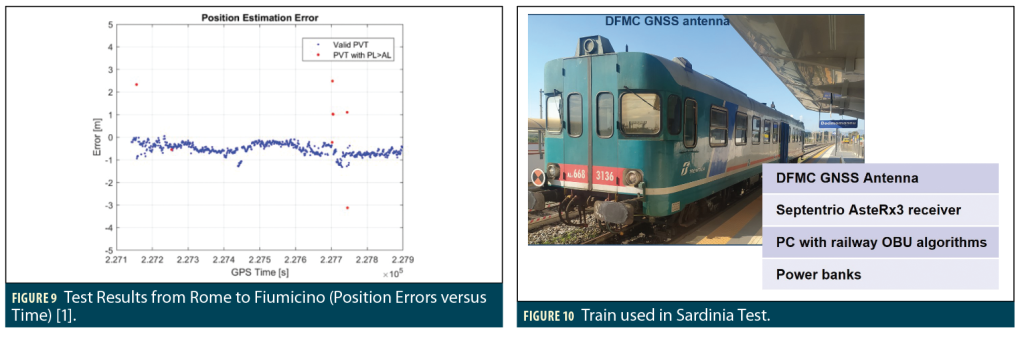
Figure 8 uses the Stanford triangle diagram to compare along-track position errors (on the x-axis) with the protection levels (PLs) computed by the OBU as bounds on this error to the GNSS THR of 10-6 per hour for this application under SL 1 in Table 1. Figure 9 shows the same position error data as a function of time during the drive from Rome to Fiumicino airport. These plots show the actual position error did not exceed about 1.25 meters for time epochs that were available, where “available” means the calculated protection level did not exceed the 5-meter alert limit for SL 1 in Table 1 (PL and AL are known to the OBU in real time and are one means of protecting integrity). As shown in Figure 8, eight epochs had PLs exceeding 5 meters, shown as red points in Figure 9. The errors observed during these unavailable epochs were significantly larger than those of the available epochs, reaching as high as 3 meters. However, because the PLs in these cases were above 5 meters, integrity would have been maintained (error ≤ PL) even if the availability determination (PL ≤ AL) were ignored.
In November 2022, the passenger train shown in Figure 10 was equipped with a prototype rail OBU and used to collect HELMET PoC measurements along the line from Cagliari to Decimomannu in southern Sardinia shown in Figure 11. This allowed HELMET to be tested in an operational rail environment.
The position errors estimated during this experiment look much like those obtained from the automobile along the Rome-to-Fiumicino highway. Figure 12 shows the Stanford triangle diagram for this test and Figure 13 shows the along-track position error versus time. The position errors in this rail test were slightly larger than those along the highway but did not exceed 2.2 meters. The along-track protection levels on the y-axis in Figure 12 were also similar to those observed along the highway. Only two out of 1,349 epochs in the rail test had protection levels exceeding the alert limit (and thus unavailable). However, note the alert limit shown in Figure 12 is 12 meters to correspond to the existing 1-D along-track requirement for ERTMS. The alert limit in Figure 9 for the automobile test is 5 meters. If it had been set to 12 meters, none of the epochs would have been unavailable.
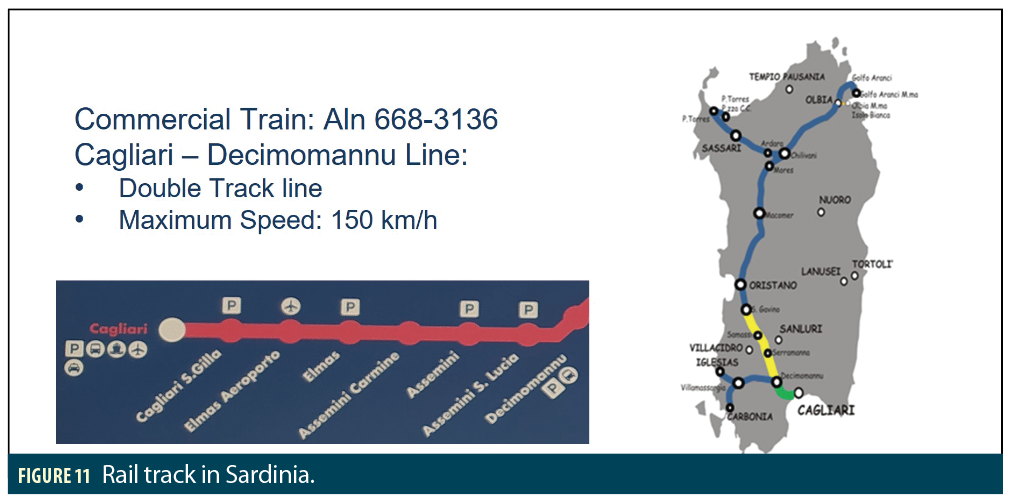
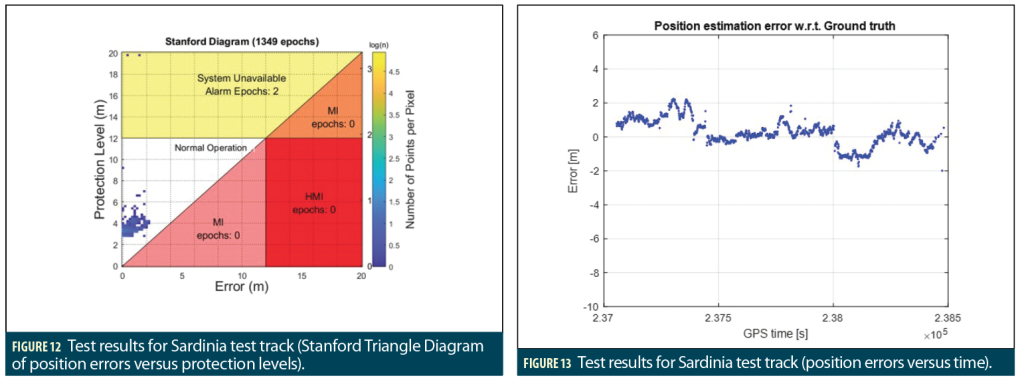
Summary
This article describes HELMET as an example of a single GNSS augmentation architecture that includes multiple service levels and multiple types of GNSS corrections and integrity information to serve multiple user classes and different requirements within each class. HELMET includes two tiers of GNSS augmentation, with multiple forms of corrections and integrity information included within the second tier (e.g., code-phase, PPP and RTK corrections). HELMET represents a practical, cost-effective approach for rail, automotive and UAV applications. HELMET illustrates how multiple forms of GNSS augmentation can be combined to support many different classes of GNSS and navigation users without requiring individual, specialized systems for each one.
Acknowledgments
The authors would like to thank the team of engineers at RadioLabs and the external partners of HELMET who developed and performed the HELMET prototype tests. They would also like to thank Dr. Daniel Lopour from EUSPA for his technical and programmatic support and Rete Ferroviaria Italiana SpA (RFI) and Azienda Nazionale Autonoma Delle Strade (ANAS) for their help in developing requirements for rail and automotive applications. The HELMET project was developed under contract 870257 from EUSPA.
References
[1] F. Rispoli, A. Vennarini, et al., “On the GNSS augmentation services for the ERTMS train control and
Connected Car applications: technical synergies and opportunities,” Proceedings of ION GNSS+ 2022.
Denver, CO, Sept. 2022, pp. 1514-1528.
https://www.researchgate.net/publication/364115778_On_the_GNSS
_augmentation_services_for_the_ERTMS_train_control_and_Connected_Car_applications_technical_sy
nergies_and_opportunities
[2] A. Neri, F. Rispoli, P. Salvatori, “The Perspective of Adopting the GNSS for the Evolution of the
European Train Control System (ERTMS): A Roadmap for a Standardized and Certifiable Platform,
”Proceedings of ION GNSS+ 2015. Tampa, FL, Sept. 2015, pp. 542-552.
https://www.ion.org/publications/abstract.cfm?articleID=12903
[3] C. Stallo, A. Neri, et al., “GNSS Integrity Monitoring for Rail Applications: Two-Tiers Method,” IEEE
Transactions on Aerospace and Electronic Systems. Vol. 55, No. 4, Aug. 2019, pp. 1850-1863.
https://doi.org/10.1109/TAES.2018.2876735
[4] S. Lo, S. Pullen, et al., “Projected Performance of a Baseline High Integrity GNSS Railway
Architecture under Nominal and Faulted Conditions,” Proceedings of ION GNSS+ 2017. Portland, OR,
Sept. 2017, pp. 2148-2171. http://web.stanford.edu/
group/scpnt/gpslab/pubs/papers/Lo_IONGNSS_2017_Railway.pdf
[5] T.G.R. Reid, A. Neish, B. Manning, “Localization & Mapping Requirements for Level 2+
Autonomous Vehicles,” Proceedings of the 2023 International Technical Meeting (ITM). Long Beach, CA,
Jan. 2023, pp. 107-123. https://doi.org/10.33012/2023.18634
Authors
Francesco Rispoli
is the General Director of RadioLabs and the Manager of the National Cluster of Transports. From 2011 to 2020, he was with Hitachi Rail, responsible for satellite operations, and was previously with Telespazio and Alenia Spazio. He received a degree in Electronic Engineering from the Polytechnic of Turin in 1978 and a Master on Applied Electromagnetism from the University La Sapienza in Roma in 1980.
Alessandro Neri
is a full professor in Telecommunications at Roma Tre University. Since 2009, he is the President of RadioLabs, a not-for-profit research center based on a partnership between universities and industrial companies. Since November 2022, he is the Vice Rector for Innovation and Technology Transfer at Roma Tre University. He is the chairman of WG-2 of the RTCM SC-134 Special Committee. His research activity has mainly been focused on information theory, signal and image processing, and location and navigation technologies.
Alessia Vennarini received a Master’s Degree in Information and Communication Technology Engineering from University Roma Tre in 2011. In 2012 she joined the RadioLabs Research Center as a GNSS expert. Since then, she has been involved in European and national projects concerning the use of GNSS for train traffic management and connected road vehicles.
Arianna Persia received a Master’s Degree in Telecommunication Engineering from the University of L’Aquila (Italy). In 2020, she received a Ph.D. in Information and Communication Technologies in the Department of Information Engineering, Computer Science and Mathematics of the University of L’Aquila. Since 2020, she has been a researcher at the RadioLabs Consortium, where she is currently involved in European and national projects in the field of satellite navigation and connected road vehicles.
Roberto Capua
is an Electronic Engineer with 25 years of experience in GNSS applications along with Research and Development for Public and Private Organizations. Currently, he is responsible for GNSS R&D for SOGEI, the technological partner of the Ministry of Economy and Finance of Italy. He is the Secretary of the Galileo Services Association and the Chairman of the RTCM SC-134 Committee “Integrity for GNSS-Based High Accuracy Applications.”