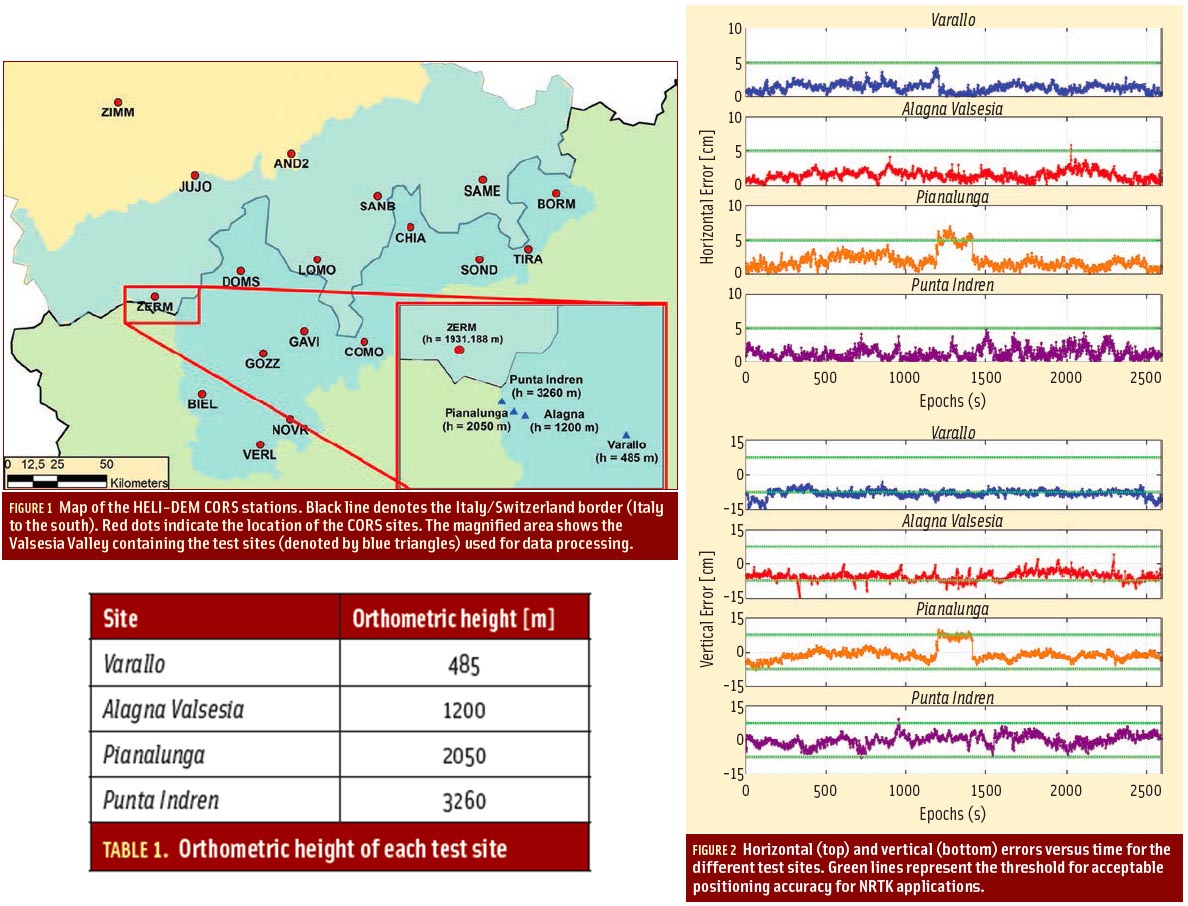
Ionospheric scintillations are rapid temporal fluctuations in both amplitude and phase of trans-ionospheric GNSS signals caused by the scattering of irregularities in the distribution of electrons encountered along the radio propagation path. The occurrence of scintillation has large day-to-day variability. The most severe scintillations are observed near the poles (at auroral latitudes) and near the equator (within ± 20 degrees of geomagnetic equator).
Ionospheric scintillations are rapid temporal fluctuations in both amplitude and phase of trans-ionospheric GNSS signals caused by the scattering of irregularities in the distribution of electrons encountered along the radio propagation path. The occurrence of scintillation has large day-to-day variability. The most severe scintillations are observed near the poles (at auroral latitudes) and near the equator (within ± 20 degrees of geomagnetic equator).
Equatorial amplitude scintillation affects both code and carrier tracking and degrades pseudorange and carrier phase measurements. Deep amplitude fades over sufficient duration can cause loss of lock in both code and carrier tracking within a GNSS receiver. Equatorial phase scintillation adversely affects the operation of a receiver’s phase lock loop (PLL) and leads to carrier cycle slips, navigation data bit errors, and complete loss of carrier lock.
Usually the level of scintillation can be characterized by the S4 amplitude scintillation index; the higher the S4 index, the more severe the scintillation. Details for computing the S4 index can be found in the Additional Resources section at the end of this article. Carrier phase tracking is very sensitive to scintillation due to a PLL’s stringent tracking threshold. Therefore, one solution for the scintillation problem is to employ a frequency lock loop (FLL) to replace a PLL for carrier tracking, due to its better robustness to signal attenuation and signal dynamics. However, many GNSS applications require (multi-frequency) carrier phase measurements, which a FLL cannot provide.
Despite the challenge of phase tracking under strong equatorial scintillation, several signal processing techniques can be used to improve the carrier tracking robustness. The common ones are FLL-assisted-PLL, adaptive-bandwidth PLL, and data stripping/aiding.
Given its robustness, using a FLL to aid a PLL seems like a wise approach. This combined carrier-tracking loop is called FLL-assisted-PLL. In a typical implementation, a FLL and a PLL operate in parallel to jointly control the carrier numerical controlled oscillator (NCO). The overall performance of such a FLL-assisted-PLL falls between that of a standalone FLL and a standalone PLL, depending on the loop noise bandwidths.
An adaptive-bandwidth PLL is another popular option for carrier tracking under scintillation. The use of a wide PLL bandwidth can help maintain phase tracking during periods of phase scintillation by tracking a rapidly changing phase. On the other hand, a narrow PLL bandwidth is desirable to tolerate amplitude scintillation with the ability to track at low carrier-to-noise–density (C/N0) ratios. The adaptive-bandwidth PLL is typically implemented by a Kalman filter with a time-varying Kalman gain based on the C/N0 conditions, because the Kalman filter explicitly models the receiver clock and optimally adapts its band-width based on C/N0.
It is well-known that a pure PLL discriminator provides an improved signal tracking threshold by up to six decibels compared to a Costas discriminator. Modernized GNSS signals provide a pilot (data-less) component and a data component. Although tracking only the pilot component can bring a three-decibel loss due to the power sharing between the data and pilot components, a net gain of three decibels still is achieved due to the pure PLL. Therefore, tracking the pilot component of modernized GNSS signals (e.g., GPS L2C and L5) is recommended in scintillation environments.
For GNSS signals that do not have a pilot component, one can still use a pure PLL discriminator with data stripping. For example, for the GPS L1 C/A signal, after storing the data bits for the entire navigation message, or with external aiding, it is possible to predict the navigation data bits until the navigation message changes. This is so-called data stripping. With the help of data stripping, one can use a pure PLL discriminator instead of a Costas discriminator in the short term for better carrier tracking under scintillation.
Multi-Satellite, Multi-Frequency Solutions
The techniques that we have discussed thus far focus on improving the carrier tracking in a single-frequency scalar-based receiver. Carrier tracking under scintillation can be improved by using multi-satellite and multi-frequency aiding. This is because scintillation rarely occurs on all visible satellites simultaneously on account of the isolated nature of electron irregularities mentioned in the introduction.
Furthermore, scintillation is carrier frequency–dependent. Both amplitude and phase scintillation levels has an inverse relation with the signal carrier frequency. The lower the carrier frequency, the stronger the scintillation is. In other words, if the same GPS signal was broadcast on L1, L2, and L5 frequencies at the same power, scintillation will most likely affect the L2 and L5 signals more than the L1 signal.
For the benefits of both frequency diversity and satellite diversity, it is important to implement the multi-satellite and multi-frequency aiding in a multi-frequency GNSS receiver. A typical implementation of such a processing architecture — herein referred to Shared-Architecture A — is shown in Figure 1 (see inset photo, above right, for all figures).
For the purpose of a better illustration, only the processing for GPS L1 C/A and L2C signals is shown in the figure. Each satellite has multiple channels for tracking signals on different frequencies. Each channel has a Doppler removal and correlation (DRC) unit, a local signal generator unit, and a PLL.
In this architecture, the key component is the multi-frequency vector delay and frequency lock loop (VDFLL). It receives the correlation from all channels and tracks the code and frequency of all signals from all satellites jointly. Regardless of the implementation variations, a VDFLL should include a local PVT (position, velocity, and time) engine that accepts multi-frequency code and Doppler measurements. The most common choice of this local PVT engine is an extended Kalman filter.
The VDFLL provides two feedback signals for each channel. One is the code phase error or the code Doppler to control the code NCO in the local signal generator, and the other is the carrier Doppler to aid the PLL in each channel. These code phase errors and the carrier Doppler values are derived from the local PVT engine.
With the carrier Doppler aiding from the VDFLL, the PLL in each channel only needs to track the residual Doppler (i.e., that induced by scintillation). The main implementation challenge for Shared-Architecture A is incorporating a reliable multi-constellation, multi-frequency PVT engine, as implementation of multi-frequency aiding and multi-satellite aiding depend upon it.
Figure 2 presents an alternative processing architecture, named Shared-Architecture B. Compared to Shared-Architecture A, this architecture employs a delay lock loop (DLL) for code tracking in each channel, and the multi-frequency VDFLL is replaced by a single-frequency VFLL and a code phase estimator.
In this architecture, DLLs are carrier-aided by PLLs to provide low-noise pseudorange measurements. As the carrier aiding from PLLs are fused by a VFLL, the multi-satellite aiding still propagates to DLLs indirectly from the PLLs in L1 C/A channels, even though a vector delay lock loop (VDLL) is not used in this case. Instead of being used to control the code NCOs, the code phase estimates derived from the single-frequency PVT engine are applied to detect loss of code lock on L1 C/A signals and to steer the DLLs when loss of code lock does occur.
In Shared-Architecture B, because the L1 C/A signal is more resistant to equatorial scintillation than the L2C signal, the L2C PLLs are aided by the L1 C/A PLLs. Since the carrier Doppler from the L1 C/A channels is already fused by the VFLL, the L2C PLLs benefit from the satellite diversity as well. In the L2C channels, similar to the L1 C/A channels, the carrier-aided DLLs are used for code tracking. The estimated L2C code phases based on the L1 C/A timing information are only used for detecting the loss of code locks and for code steering after loss of locks.
Shared-Architecture B also has the advantage of implementing multi-satellite aiding and multi-frequency aiding separately. For example, if only the multi-frequency aiding mode is needed, the receiver can simply disable the VFLL aiding and keep the L1-to-L2 aiding, which Shared-Architecture A cannot.
For signal acquisition, both architectures only acquire the signal on a single frequency (referred to here as the master frequency signal), because the carrier Doppler and timing information of the master frequency signal can directly estimate the code phase and carrier Doppler parameters of other frequency signals. In order words, the timing information and carrier Doppler from the master frequency signal can be used to directly initialize the code and carrier tracking loops of other frequency signals. The typical choice of the master frequency signal is the GPS L1 C/A signal, due to its shorter ranging code and stronger scintillation resistance compared to others.
We implemented Shared-Architecture A and Shared-Architecture B in a multi-constellation, multi-frequency GNSS software receiver. We will only present here the results of the Shared-Architecture B with the intermediate frequency (IF) data affected by equatorial ionospheric scintillation, but results with Shared-Architecture A were similar.
Data was collected in Rio de Janeiro during a research project established between the Brazilian Institute of Geography and Statistics (IBGE), the University of the State of Rio de Janeiro (UERJ), and the Position, Location And Navigation (PLAN) Group at the University of Calgary, to investigate the effects of equatorial ionospheric scintillation on GNSS signals during the current solar maximum.
Figure 3 shows the percentile of valid L1 C/A and L2C phase observations with the Shared-Architecture B and a standard architecture (without using multi-frequency and multi-satellite aiding). Valid phase observations are defined as those where the carrier phase observations have a phase lock indicator (PLI) value larger than 0.6 (see Additional Resources) and no cycle slips are detected. The results are based on eight hours of data using two-hertz measurements.
As reflected in the figure, due to the benefits of multi-frequency/multi-satellite aiding, Shared-Architecture B provides significantly more valid measurements than a standard architecture over the entire S4 range, especially for the L2C carrier phase. This demonstrates the importance of the multi-frequency/multi-satellite aiding for a multi-frequency GNSS receiver.
In conclusion, despite the challenge of carrier tracking under strong equatorial scintillation, the performance of carrier tracking can be improved via modern signal processing techniques. Due to the natural characteristics of scintillation, multi-frequency/multi-satellite aiding benefits multi-frequency carrier tracking under equatorial ionospheric scintillation. The improved performance of the proposed multi-frequency/multi-satellite aiding was shown with IF data collected in Brazil.
Additional Resources
For equatorial scintillation
Doherty, P. H., and S. H. Delay, C. E. Valladares, and J. A. Klobuchar, “Ionospheric Scintillation Effects in the Equatorial and Auroral Regions,” Proceeding of ION GPS-2000, Salt Lake City, Utah, USA, September 2000
For S4 index calculation and PLI calculation
Van Dierendonck A.J., and J. Klobuchar and Q. Hua, “Ionospheric Scintillation Monitoring Using Commercial Single Frequency C/A Code Receivers,” Proceedings of the 6th International Technical Meeting of Satellite Division of the Institute of Navigation (ION GPS 1993), pp. 1333-1342, September 1993
Van Dierendonck, A. J., “GPS Receivers,” in Global Positioning System: Theory and Applications, Vol. 1, AIAA, Washington, DC, pp. 330–433, 1996
For FLL-assisted-PLL and adaptive-bandwidth PLL
Ward, P. W., “Performance Comparison between FLL, PLL and a Novel FLL-Assisted-PLL Carrier Tracking Loop Under RF Interference Conditions,” Proceedings of ION GNSS 1998, pp. 783-795, September 1998
Humphreys, T. E., and M. L. Psiaki, B. M. Ledvina, and P. M. Kintner, Jr., “GPS Carrier Tracking Loop Performance in the Presence of Ionospheric Scintillations,” Proceedings of ION GNSS 2005, Long Beach, California, USA, September 2005
For vector tracking
Lashley, M., and D. Bevly , “GNSS Solutions: How do vector delay lock loops predict the satellite signals?” Inside GNSS, September/October 2012
For GSNRx
http://plan.geomatics.ucalgary.ca/project_info.php?pid=27, including the following available on that website:
Petovello, M. G., and C. O’Driscoll, G. Lachapelle, D. Borio, and H. Murtaza, “Architecture and Benefits of an Advanced GNSS Software Receiver,” Journal of Global Positioning Systems, vol. 7, no. 2, pp. 156–168, 2008