The startup plans to launch its first demonstration mission on SpaceX’s Transporter 5 in May, with the goal of displaying high-quality commercial PNT LEO signals from a payload suitable for small satellite deployment.
By Dr. Tyler Reid
Global Navigation Satellite Systems (GNSS) enabled revolutionary advancements in countless aspects of the 21st century. GNSS is an invisible utility that provides navigation and timing services to more than 6.4 billion devices [1]. This supports a diversity of applications including mobile phones, the power grid, telecommunications, agriculture, financial transactions and transportation.
In recent years, these industries have seen substantial advancement and are now demanding more from Position, Navigation and Timing (PNT) than what GNSS today can provide. Specifically, they seek improved resilience, accuracy and security as highlighted in recent mandates from U.S. Presidential Executive Orders [2,3]. Perhaps most demanding are the requirements for autonomous systems like SAE Level 4 self-driving cars, suggested by some to require position protection levels at 30 cm with 99.999999% reliability, or one failure in a billion miles driven [4]. Reliable decimeter location unlocks a new generation of applications, enabling humans and machines to interoperate in shared physical spaces.
The question is, how should our PNT infrastructure evolve to support these applications on the horizon?
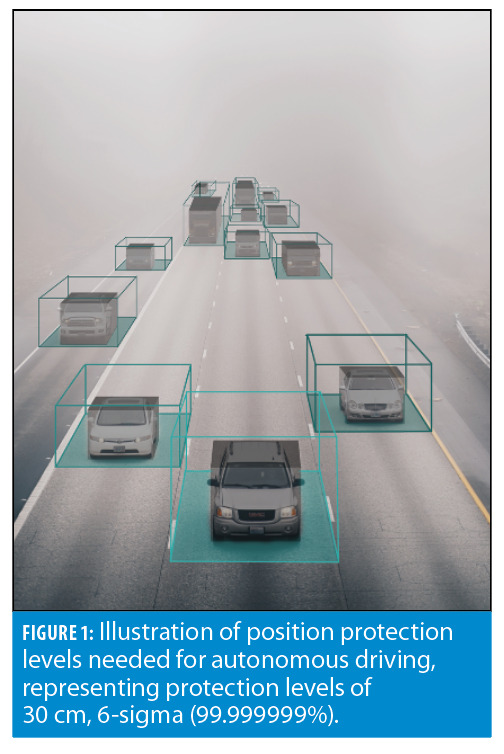
GNSS in Autonomy
To understand the role of satellite navigation in autonomy, we will begin with a look at how satellite navigation is used today in autonomous systems. Primarily, SAE Level 4 prototype self-driving cars rely on LiDAR and 3D map matching for localization. Other sensors also play a role, such as IMUs, cameras, radar, ultrasonics, and, of course, GNSS, though it is used mostly for high level routing.
This choice goes all the way back to design decisions made during the early DARPA autonomous driving challenges. In the 2004 DARPA Grand Challenge, GPS had been available to civil users for only a handful of years, so capability and user equipment were somewhat limited. On the day of the race, several teams experienced GPS jamming by the jumbotron, cementing GPS as unreliable to some who are now captains of the autonomous industry [5-7].
The choice of whether to use LiDAR also has to do with where these vehicles are operating, which today is largely in major cities and in good weather. Cities give sufficient 3D information for a position fix from map matching. In contrast, LiDAR tends to struggle with open flat featureless areas where there’s a lack of unique attributes to gain confidence in a position solution. Furthermore, sensors like LiDAR are subject to scattering and absorption in adverse weather conditions [8-11].
The approach taken by the automotive industry toward solving the localization problem is redundancy. To achieve the sought 99.999999% reliability in autonomous localization systems (often referred to as ASIL D), one approach is to decompose the system into two or more independent ASIL B (99.9999%) systems. As shown in Figure 2, there are largely two methods of localization employed today, the previously mentioned ‘relative’ approach that localizes to a high-resolution map using cameras, LiDAR, or other vehicle-relative sensors, and the ‘absolute’ approach that relies on precision GNSS [12].
Satellite navigation works well in open skies (like on highways), which is where LiDAR and computer vision can be less reliable. Likewise, sky obstructions that block GNSS are targets for LiDAR and vision-based localization, hence their complementary nature. Satellite navigation further offers a common vantage point at a world scale, enabling a common reference frame for data sharing to establish situational awareness at the city level. Building such a complete picture is yet another step toward closing the safety case in autonomy. GNSS will continue to be present in automotive systems, but how can it evolve to ultimately meet the stringent requirements of SAE level 4?



Designing Commercial Satnav
One approach is to tackle the challenge of PNT infrastructure commercially, bringing new capabilities to support specific commercial needs. The Earth observation and telecommunications industries have seen disruption with commercial space infrastructure, particularly in recent years.
In connectivity, SpaceX, OneWeb and others are all building constellations of thousands or tens of thousands of satellites to provide global broadband. SpaceX has more Starlink satellites in orbit than there were total operational satellites just 5 years ago. Starlink satellites can be launched 60 at a time, nearly an Iridium constellation’s worth of satellites.
In geospatial data, commercial Earth observation constellations from the likes of Planet and Spire are providing a better geospatial view of our planet.
These are the so-called mega-constellations, and they represent the new space economy enabled by lower launch costs and new satellite production techniques. As a result, in 5 years the number of total operational satellites has increased by threefold. Most of this growth has been in low Earth orbit (LEO), with a fivefold growth in 5 years. Both Earth observation and telecommunication saw a transition from government-only control to the commercial sector, but we have not yet seen this in navigation.
This begs the question: How would you build satellite navigation today for commercial users? To contrast commercial user requirements with those of government focused GNSS, consider Table 1, which compares select requirements where we will use GPS as an example.
First is the user group, which is commercial versus government users. Next is accuracy. GPS was designed famously to ‘drop 5 bombs in the same hole[14].’ Today, commercial users want to keep their car in its lane. In terms of availability, both want global performance, but commercial users are more focused on population centers than government users. Both user groups are concerned with interference, but one with state-level actors and the other with smaller personal privacy devices. Finally, commercial users are focused on mass-market deployment of user equipment.
Consider Figure 5, which describes the design decisions that arise from GPS requirements. We start with ‘drop 5 bombs in the same hole,’ which translates to 10 meters RMS positioning [15]. Next is positioning technique, where a broadcast system is desired because it keeps the user equipment simple and stealthy. How? No communication is required back to the satellite. It also supports an unlimited number of users. For the signal, CDMA was chosen for efficient use of spectrum and jam mitigation.
Now, we come to military requirements, the first of which is that the space segment must be radiation hardened to survive and operate through a nuclear strike [16]. This is the most stringent radiation hardening level, a level IVa out of a 4-point scale as defined by the Institute of Electrical and Electronics Engineers (IEEE) standards [17], leading to bespoke and highly specialized satellite hardware. It is noteworthy that the GPS orbits are in the outer Van Allen radiation belts, yielding natural levels of radiation higher than both LEO (Level I/II) and GEO (Level III), defined as Level IV by the IEEE [17,18].
The system must also operate autonomously without access to the ground for at least two weeks in the event the ground segment is unavailable or
incapacitated [19]. This is where the atomic clock requirements on the satellites come from; they’re needed to maintain holdover synchronization between satellites operating autonomously.
The next military requirement is a daily domestic uplink to the satellite. This rules out geosynchronous orbit because it denies access to certain satellites that would remain over other parts of the world.
So, what orbit do you choose? The tradeoff was the satellites had to be radiation hardened, have atomic clocks and be built in the 1970s and 1980s. The result was MEO won over LEO. The satellites were going to cost about the same due to radiation hardening and clock requirements, but 10x fewer satellites were needed in MEO to obtain the same coverage. Launch cost won over build cost. This is very much the model that has been followed since GPS, and is why other government GNSS constellations look similar in many ways. Ultimately, they all share similar government requirements.
What might you do differently for a commercial system? We can start by removing government-specific requirements. First, we can leverage commercial ground stations as a service, as these have become common in the new space era. Next, we assume these mission-critical commercial ground services have very high up time. We can remove the two-week holdover requirement and as a result remove atomic clocks from the satellites, leading to lower cost and complexity clocks in the space segment. Next, we can remove the stringent radiation hardening requirement, instead making satellites radiation tolerant to their space environment.
This leads us to the orbit. We want an orbit that offers (1) higher receive power for users for resilience to interference (which pushes the system closer to Earth), (2) rapid motion across the sky for fast PPP convergence to centimeter positioning (also pushes closer), (3) and a low-cost space segment, which in today’s ecosystem means leveraging the launchers, satellite components, and supply chains of the LEO mega-constellations.
In terms of performance, fast PPP convergence means convergence to better than 10 cm on the timescale of a minute or less without local atmospheric corrections, even with traditional PPP. This is powerful because it brings precision globally without dense local infrastructure. So, both from a performance and economics standpoint, these factors are pushing us toward low Earth orbit.
The result is a commercial satellite navigation constellation in LEO, with low-cost satellites produced in volume using largely off the shelf space-capable components. This is not to say you replace space-grade components with mass market ones, just that the mega-constellations’ ecosystem has resulted in a healthy space-capable component supply chain. In such a system configuration, constellation orbit determination and clock synchronization can be achieved through inter-satellite links in a way that is GNSS independent. This architecture allows for atomic timescales to be placed in specific nodes in the network, either on the ground or in space, in lieu of on every satellite.
Lastly, new LEO signals are not bound by legacy, so there can be inclusion of encryption and data authentication for civil users. A commercial LEO layer such as this can add capability that is both reprogrammable on-orbit for rapid updates and that can evolve quickly, on a 5-year timescale, to keep pace with commercial demands. It is much more difficult to do this with government GNSS services because they are already supporting billions of devices and hence there is a big inertia to large changes. We can see the complimentary nature of government MEO systems and commercial LEO PNT.


New Space Commercial LEO PNT
This approach to commercial LEO PNT is being taken by Xona Space Systems. Xona, now a VC-backed startup, was founded in 2019 around the idea that modern technology has the potential to benefit people in all walks of life, but to do so it needs to operate safely in any conditions, anywhere on Earth. To enable this, Xona is working to provide resilient and accurate PNT services: satellite navigation for the age of autonomy [20]. Xona is challenging the assumption that the GNSS space segment is fixed and out of reach of commercial entities.
Xona was founded by a group of eight who first met more than a decade ago in Stanford University’s Aero Astro Department. After graduation, some went on to work in the aerospace industry at the likes of SpaceX, Blue Origin and Maxar, while others pursued further graduate studies in the GPS Research Lab. Reuniting in 2019, Xona is now headquartered in San Mateo, California, with additional offices in Vancouver, Canada, and London, UK.
Now a global team of 30, Xona is gearing up to launch its first demonstration LEO PNT missions. Named Huginn and Muninn, this pair sets out to demonstrate high quality commercial PNT LEO signals from a payload suitable for small satellite deployment. In Norse mythology, Huginn (meaning thought) and Muninn (meaning memory) were the Ravens of the God Odin who would fly around the earth every day to bring information back to Odin. Huginn and Muninn are pathfinders for Xona, circling the globe and returning to Xona’s offices with new navigation information every day. The first, Huginn, is scheduled for launch in May on SpaceX’s Transporter 5.
Huginn will broadcast navigation signals in L-band and C-band, providing ranging and navigation data, including corrections for GNSS. It is expected that the rapid geometry change from even just one spacecraft in LEO can greatly accelerate the convergence of GNSS PPP, cutting convergence time in half or more.
With more LEO satellites in view, convergence time is expected to improve by fivefold or more without the need for local infrastructure for rapid PPP convergence, bringing forth a solution that is truly global. These experimental PNT signals will also offer security to mitigate the threat of spoofing, using NIST standardized Advanced Encryption Standard (AES) algorithms and Elliptic Curve Digital Signature Algorithm (ECDSA) for code and data authentication. Huginn is software re-programmable, offering a flexible payload on orbit, enabling Xona to test additional advanced capabilities as well.
A signal Interface Control Document (ICD) has been created for the demonstration mission that describes the signals, modulation and data structures in more detail and is available upon request. This has been shared with several partners to aid in prototype receiver development.



Looking Forward
The Huginn and Muninn demonstration missions are the first step toward deployment of Pulsar, Xona’s commercial PNT service. In its ultimate form, this embodies a LEO constellation of several hundred satellites, delivering GPS-level satellite visibility and geometry and full PNT services that are precise and secure. This brings together high accuracy and resilience at the level needed to support applications like self-driving vehicles.
To deploy the service, a phased roll out plan has been devised with different levels of service planned along the way. Phase 1 is deployment of ~40 satellites, which offers a 1-in-view GNSS enhancement service over population centers (mid latitudes). This brings GNSS correction services coupled with LEO geometry change for rapid PPP convergence to centimeter positioning. This adds an additional ranging signal from a fifth constellation that is resilient and secure. Furthermore, it enables time transfer for stationary users, supporting critical infrastructure.
Phase 2 expands the constellation to ~70 satellites, which offers the same service as Phase 1, globally. This fills in gaps over the poles and bolsters satellite numbers over mid-latitudes. These are steppingstones toward the Phase 3 deployment of several hundred satellites, which brings full positioning services online that are precise and secure.
Figure 12 shows a global map of the expected satellite visibility for terrestrial users of the Phase 3 Xona Pulsar constellation. Figures 13 and 14 show the cumulative distribution of satellite visibility and dilution of precision, respectively. This yields a median number of satellites in view of around 15 with a VDOP of 1.2 in population centers. It shows the Xona Pulsar constellation as designed can offer GPS-level performance in visibility and geometry, supporting users at all latitudes.
The concept represents a new space approach to commercial satellite navigation, one architected to meet the PNT needs of demanding critical applications, including autonomous vehicles. It leverages the ecosystem that has enabled the mega constellations including SpaceX, OneWeb, Telesat and Amazon.
These factors comprise orders of magnitude in cost reduction in access to space with reusable launch vehicles along with new techniques in high volume satellite production. The latter has led to the added benefit of a healthy supply chain of off-the-shelf space-capable components. The commercial constellation is designed for LEO to use mega-constellation satellite buses, components and launch systems intended for that regime and to provide higher performance to the end user.
Satellites are designed for flexibility and with a 5-year lifetime, allowing for faster refresh cycles to keep pace with ever increasing commercial PNT demands. To achieve the required performance and reliability, Xona introduces a distributed architecture that leverages an interconnected lightweight space segment along with enterprise ground station services to achieve GNSS independence. It combines familiar signals elements with novel ones for new capability and added resilience, including stronger, spectrally diverse signals with encryption and authentication for civil users.



References
(1) European Global Navigation Satellite Systems Agency, “GNSS Market Report, Issue 6,” 2019. doi: 10.2878/031762.
(2) U.S. Department of Transportation, “Executive Order on Strengthening National Resilience through Responsible Use of Positioning, Navigation, and Timing Services|U.S. Department of Transportation,” Feb. 12, 2020. https://www.transportation.gov/pnt/executive-order-strengthening-national-resilience-through-responsible-use-positioning (accessed Feb. 08, 2021).
(3) S. Erwin, “Policy directive on GPS issued in closing days of Trump administration—SpaceNews,” Space News, Jan. 16, 2021. https://spacenews.com/policy-directive-on-gps-issued-in-closing-days-of-trump-administration/ (accessed Feb. 08, 2021).
(4) T. G. R. Reid et al., “Localization Requirements for Autonomous Vehicles,” SAE Int. J. Connect. Autom. Veh., vol. 2, no. 3, pp. 12-02-03–0012, Sep. 2019, doi: 10.4271/12-02-03-0012.
(5) N. Joubert, T. G. R. Reid, and F. Noble, “Developments in Modern GNSS and Its Impact on Autonmous Vehicle Architectures,” 2020, [Online]. Available: https://arxiv.org/abs/2002.00339.
(6) C. Urmson et al., “High Speed Navigation of Unrehearsed Terrain: Red Team Technology for Grand Challenge 2004,” 2004. doi: CMU-RI-TR-04-37.
(7) L. D. Burns and C. Shulgan, Autonomy: The Quest to Build the Driverless Car—And How It Will Reshape Our World. Scientific American, 2018.
(8) S. Sundararajan and I. Zohdy, “Vehicle Automation and Weather: Challenges and Opportunities, US DOT Report: FHWA-JPO-17-494,” Washington, DC , 2016. [Online]. Available: https://rosap.ntl.bts.gov/view/dot/32494/dot_32494_DS1.pdf.
(9) S. Michaud, J.-F. Lalonde, and P. Gigù Ere, “Towards Characterizing the Behavior of LiDARs in Snowy Conditions,” 2015.
(10) M. Kutila, P. Pyykönen, W. Ritter, O. Sawade, and B. Schäufele, “Automotive LIDAR sensor development scenarios for harsh weather conditions,” in IEEE Conference on Intelligent Transportation Systems, Proceedings, ITSC, Dec. 2016, pp. 265–270, doi: 10.1109/ITSC.2016.7795565.
(11) R. Heinzler, P. Schindler, J. Seekircher, W. Ritter, and W. Stork, “Weather Influence and Classification with Automotive Lidar Sensors,” in IEEE Intelligent Vehicles Symposium (IV), Aug. 2019, pp. 1527–1534, doi: 10.1109/ivs.2019.8814205.
(12) N. Joubert, T. G. R. Reid, and F. Noble, “Developments in Modern GNSS and Its Impact on Autonomous Vehicle Architectures,” 2020, doi: 10.1109/IV47402.2020.9304840.
(13) “Satellite Database | Union of Concerned Scientists.” https://www.ucsusa.org/resources/satellite-database (accessed Apr. 21, 2022).
(14) B. W. Parkinson, “Origins, Evolution, and Future of Satellite Navigation,” J. Guid. Control. Dyn., vol. 20, no. 1, pp. 11–25, Jan. 1997, doi: 10.2514/2.4027.
(15) B. W. Parkinson and J. J. Spilker, The global positioning system: theory and applications vol. 1. 1996.
(16) J. Keller, “The evolving world of radiation-hardened electronics,” Military Aerospace Electronics, Jun. 01, 2018. https://www.militaryaerospace.com/computers/article/16707204/the-evolving-world-of-radiationhardened-electronics (accessed Apr. 22, 2022).
(17) IEEE Std 1156.4-1997 : IEEE Standard for Environmental Specifications for Spaceborne Computer Modules. IEEE, 1997.
(18) T. G. R. Reid, A. M. Neish, T. Walter, and P. K. Enge, “Leveraging Commercial Broadband LEO Constellations for Navigation,” in Proceedings of the 29th International Technical Meeting of The Satellite Division of the Institute of Navigation (ION GNSS+ 2016), 2016, pp. 2300–2314, Accessed: Sep. 20, 2018. [Online]. Available: http://web.stanford.edu/group/scpnt/gpslab/pubs/papers/Reid_IONGNSS_2016_LEO.pdf.
(19) A. Flores, “NAVSTAR GPS Space Segment/Navigation User Interfaces (IS-GPS-200 Rev. M),” El Segundo, CA, 2021. [Online]. Available: https://www.gps.gov/technical/icwg/IS-GPS-200M.pdf.
(20) T. G. R. Reid et al., “Satellite Navigation for the Age of Autonomy,” in 2020 IEEE/ION Position, Location and Navigation Symposium, PLANS 2020, Apr. 2020, pp. 342–352, doi: 10.1109/PLANS46316.2020.9109938.
Authors
Dr. Tyler Reid is a co-founder and CTO of Xona Space Systems. Previously, Tyler worked as a research engineer at Ford Motor Company in the localization and mapping group for self-driving cars. He has also worked as a software engineer at Google and as a lecturer at Stanford University, where he co-taught the GPS course. Tyler received his Ph.D. (2017) and MSc (2012) in Aeronautics and Astronautics from Stanford University, where he worked in the GPS Research Lab.